Operon Sequencing: Mechanisms and Implications
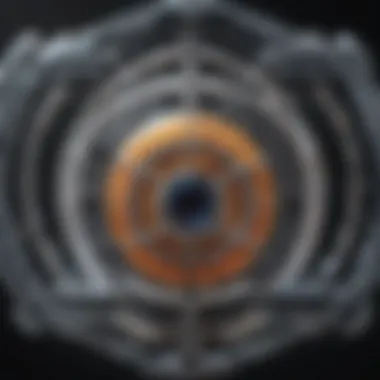
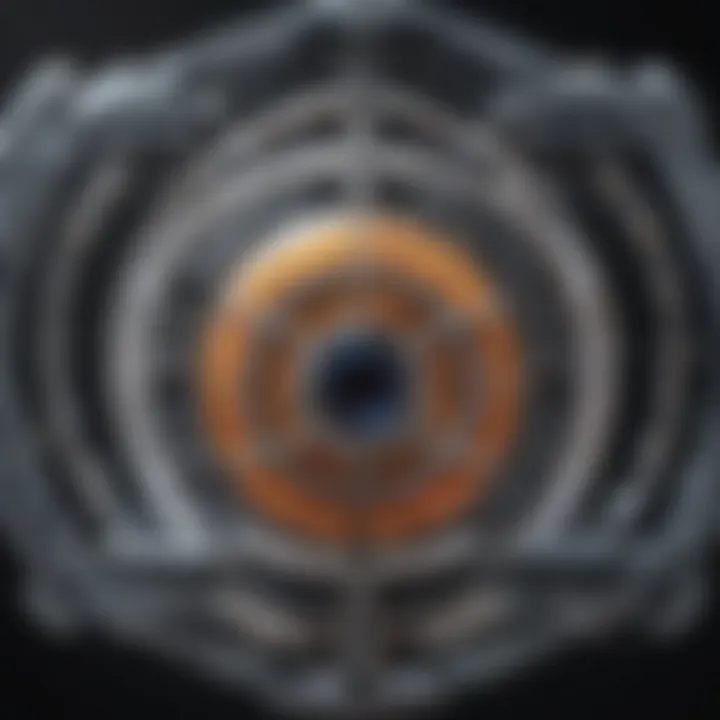
Intro
Operon sequencing represents a pivotal advancement in the understanding of genetic regulation and expression. This mechanism allows for the coordinated control of multiple genes, facilitating efficient cellular responses to environmental changes. The exploration of operons not only sheds light on the intricacies of microbial genetics but also highlights potential applications within synthetic biology. By dissecting the structural organization, sequencing methodologies, and analytical techniques used in operon research, one can appreciate the profound implications these systems hold for future genetic studies and their broader applications in biotechnology and medicine.
Article Overview
Summary of Key Findings
Operon sequencing reveals a structured approach to gene regulation. Key findings demonstrate that operons are primarily found in prokaryotic organisms, where they cluster functionally related genes under the control of a single promoter. This arrangement significantly enhances efficiency in gene expression.
Moreover, recent technological advancements in sequencing methods, such as Next-Generation Sequencing (NGS), have enabled researchers to ascertain detailed operon structures and their functional roles. This article discusses significant sequential data interpretation methodologies further enhancing the understanding of operon activities.
Research Objectives
The main objectives of this article include:
- To elucidate the structural organization of operons and their genetic contexts.
- To examine methodologies for operon sequencing, highlighting current technologies in use.
- To interpret the analytical techniques necessary for understanding sequencing data.
- To discuss the implications of operon sequencing in various biological fields, including its potential in synthetic biology.
By achieving these objectives, the article aims to provide a foundation that is essential for both seasoned researchers and newcomers to the field. Understanding operon sequencing is not just about the mechanics; it is about leveraging that knowledge to push boundaries in genetic research and application.
Key Results and Discussions
Main Findings
This article synthesizes important findings relevant to operon sequencing, such as the correlation between operon structure and function. High throughput sequencing studies have revealed that variations in operon configurations can lead to differing gene expression profiles, which is critical in adapting to environmental pressures.
Additionally, comparative genomics has identified conserved operon structures across related species, which hints at evolutionary advantages. The research indicates that operons can serve as evolutionary reservoirs, allowing organisms to adapt by horizontal gene transfer among species, thereby increasing genetic diversity.
Implications of Findings
The implications of these findings are profound. Operon sequencing not only enriches the understanding of microbial genetics but also opens avenues in synthetic biology by providing templates for designing engineered organisms. This ability to manipulate gene clusters facilitates the development of microbial strains optimized for biotechnological applications, such as biofuel production or biodegradable plastics.
Moreover, these insights contribute to the field of medicine, particularly in developing new therapeutic approaches. As operon structures are studied further, there may be opportunities to target bacterial operons for novel antibacterial drug development.
"Understanding the structure and function of operons pushes the boundaries of what can be achieved through genetic engineering strategies."
In summary, operon sequencing serves as a key player in genetic research, driving forward our comprehension of gene regulation and providing substantial ground for future innovations in biotechnology.
Intro to Operons
Operons are a fundamental concept in molecular biology, offering insight into gene regulation and expression. They serve as a model for understanding how genes work together to produce proteins and how their activity is controlled within a cell. Grasping the mechanisms of operons is critical for researchers and students as it lays the groundwork for advanced studies in genetics and biochemistry. Operons illustrate the elegance of genetic regulation; they allow bacteria to adapt quickly to changes in their environment.
The importance of operons extends into several realms of genetic research, including microbial genetics and synthetic biology. Understanding the structure and function of operons enables researchers to manipulate genetic systems and develop biotechnological applications. The implications are vast: from designing more effective antibiotics to advancing our understanding of metabolic pathways in microorganisms.
In this section, we will explore the definition of operons and the historical background that has shaped our current understanding of these genetic structures. This overview will set the stage for a deeper dive into their regulation and sequencing techniques.
Structure of Operons
Understanding the structure of operons is crucial for exploring how genes are regulated in prokaryotic organisms. Operons facilitate the coordinated expression of gene clusters, enabling organisms to respond efficiently to environmental changes. Each component plays a unique role in the operon’s functionality, impacting how genes are turned on or off. Knowing these structures helps illuminate the broader implications in fields like genetics and biotechnology.
Components of Operons
Promoter
The promoter is a vital part of an operon. It serves as the starting point for transcription, which is the first step in gene expression. The key characteristic of a promoter is its specific sequence that RNA polymerase recognizes, allowing it to bind. This process is essential as it determines when and how much of a protein is produced.
Promoters are often chosen for their ability to allow tightly regulated responses to environmental signals. The unique feature of promoters is that they can be strong or weak in their activity. A strong promoter drives high levels of transcription, while a weak one leads to lower expression. Balancing these strengths allows researchers to manipulate gene expression effectively for various applications.
However, a strong promoter can also lead to transcriptional noise, potentially overwhelming cellular machinery.
Operator
The operator functions as a regulatory region in the operon. It lies adjacent to the promoter and interacts with regulatory proteins that can either enhance or inhibit gene expression. The key characteristic of the operator is its ability to bind repressor proteins, which block transcription when bound. This selective control makes it an essential component in gene regulation.
The operator’s unique feature allows for quick responses to changes. For example, if a substrate is present, the repressor will be removed from the operator, permitting gene transcription. On the downside, this means that if the regulation is disrupted, it can lead to uncontrolled gene expression, impacting cellular function negatively.
Structural Genes
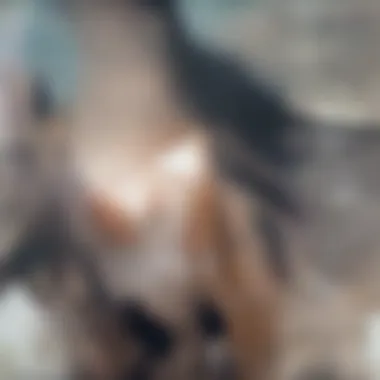
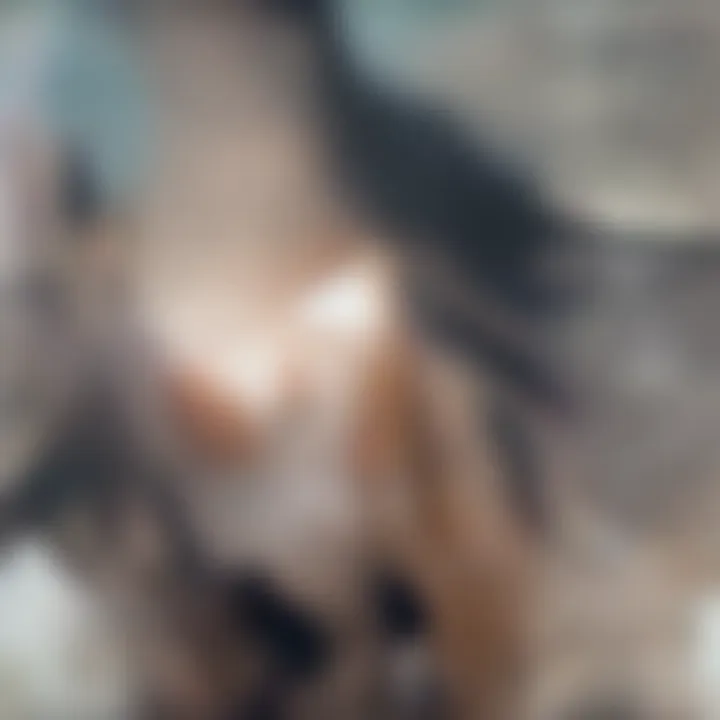
Structural genes are the actual coding regions of the operon that encode proteins. Each gene in the operon is transcribed into a single mRNA strand, allowing simultaneous expression. The key characteristic of structural genes is their organization in a linear sequence following the promoter and operator. This arrangement facilitates the coordinated expression of related functions.
One unique feature is that structural genes can vary in number across different operons. For instance, an operon may consist of multiple structural genes all involved in a specific pathway. However, this can also lead to complications. If one gene is regulated poorly, it can affect all others, leading to overall reduced efficiency.
Types of Operons
Inducible Operons
Inducible operons respond to specific signals that trigger gene expression. The classic example is the lac operon in E. coli. Its induction occurs when lactose is present, allowing the bacteria to utilize this sugar efficiently. The key characteristic of inducible operons is their ability to turn on gene expression in the presence of an inducer. This enables organisms to adapt to changes in nutrient availability.
Inducible operons are beneficial as they enable efficient and dynamic responses to environmental stimuli. However, misregulation may occur if the inducer is present excessively, causing wasted resources and energy.
Repressible Operons
Repressible operons generally function opposite to inducible ones, being activated by default and suppressed when a product is abundant. The tryptophan operon serves as a prominent example. When tryptophan levels are high, it activates the repressor, inhibiting further gene expression. The key characteristic of repressible operons is their negative feedback loop, which conserves cellular resources.
Repressible operons are popular as they showcase an efficient regulatory mechanism. However, they can also lead to a complete shutdown of essential pathways if the repressor is overly sensitive to the product levels.
Mechanism of Operon Regulation
Understanding the mechanism of operon regulation is crucial for comprehending how genes are expressed and controlled in various organisms. Operons play a significant role in the regulation of gene expression, a fundamental process that impacts cellular function and overall organismal development. The regulation mechanisms can be broadly categorized into transcriptional and post-transcriptional regulation, each with specific components that contribute to the orchestration of gene expression.
Transcriptional Regulation
RNA Polymerase Interaction
RNA polymerase is the enzyme central to the transcription process. Its interaction with the operon determines whether gene transcription will occur. The ability of RNA polymerase to bind effectively to the promoter region of an operon is crucial. This interaction is essential because it initiates the synthesis of RNA from the DNA template. One key characteristic of RNA polymerase interaction is that it can be influenced by various factors, such as the presence of transcription factors or small molecules that can enhance or inhibit binding.
The importance of RNA polymerase interaction lies in its ability to control gene expression dynamically. By modulating this interaction, cells can respond quickly to environmental changes. A unique feature of this interaction is that it can be reversible, allowing for fine-tuned control. However, if RNA polymerase fails to interact properly, the result may be inadequate transcription, limiting protein production and impairing cellular function.
Transcription Factors
Transcription factors are proteins that regulate the transcription of genes by binding to specific DNA sequences. Their role in operon regulation is vital, as they can either activate or repress the transcription process. One critical aspect of transcription factors is their ability to recruit RNA polymerase to the promoter region, facilitating transcription initiation. This recruitment process enhances the overall efficiency of gene expression.
Transcription factors are considered beneficial for this article due to their extensive involvement in gene regulation mechanisms. They introduce a layer of complexity that allows cells to respond to diverse signals. A unique feature is that transcription factors can interact with various types of signals, such as hormones or environmental cues, further regulating gene expression. However, the complexity they introduce can lead to challenges in understanding specific gene regulation pathways.
Post-Transcriptional Regulation
RNA Processing
Post-transcriptional regulation is another key mechanism influencing gene expression after transcription occurs. RNA processing involves various modifications, including capping, polyadenylation, and splicing. These modifications are crucial for the stability and functionality of the RNA molecule. A major characteristic of RNA processing is that it prepares the RNA for translation into proteins. This processing ensures that only mature and functional RNA is transported to the ribosome for translation.
This aspect of gene regulation is particularly relevant because it highlights how cellular mechanisms refine RNA before it can fulfill its role. A unique feature is the splicing mechanism, which allows for alternative splicing variants, resulting in multiple protein products from a single gene. However, the complexity of RNA processing may lead to errors, potentially resulting in nonfunctional proteins.
RNA Stability
RNA stability is the measure of how long an RNA molecule remains intact before degradation. It significantly influences gene expression levels as more stable RNA can translate into more proteins. A key characteristic of RNA stability is that it can be regulated through various mechanisms, including the action of ribonucleases, which can target specific RNA molecules for degradation.
This regulation is advantageous for the overarching topic of operon sequencing, as stable RNA serves as a better indicator of gene expression levels. Unique features of RNA stability include the involvement of specific sequences or structures within RNA that can either protect it from degradation or make it more susceptible. Despite its advantages, over-stable RNA might contribute to inappropriate protein levels, which may have detrimental effects on cell function.
The regulation of operons is a multifaceted process, involving various components that contribute to precise gene expression and control.
In summary, the mechanisms of operon regulation showcase the intricate layers of control that govern gene expression. Understanding these mechanisms is essential for advanced Genetic research, providing the foundation for manipulating gene expression in synthetic biology and other fields.
Operon Sequencing Techniques
Operon sequencing techniques are vital for understanding the genetic regulation inherent in operons. These methodologies allow researchers to explore the structure and function of genetic elements, providing insights into gene expression that is crucial for various biological applications. The sequencing process enables scientists to analyze operons in situ, revealing how they interact with regulatory components and the implications of these interactions in a biological context. Understanding these techniques is essential for advancing genetic research and applying these findings in fields like synthetic biology and microbial genetics.
Next-Generation Sequencing (NGS)
Next-Generation Sequencing, commonly referred to as NGS, revolutionizes the field of genomics by providing a scalable and efficient way to sequence entire genomes or specific regions of interest. NGS has made sequencing quicker and more affordable, leading to a surge in data generation. For operon sequencing, NGS allows for the simultaneous analysis of multiple operons, making it easier to capture variations and mutations that might affect gene regulation. The ability to generate vast amounts of data rapidly means that researchers can obtain comprehensive insights into the structural organization of operons and their functional implications. Thus, NGS is a transformative tool in the genetic research landscape, particularly in elucidating operon functions.
Methods of Operon Sequencing
Whole Genome Sequencing
Whole Genome Sequencing (WGS) is a method that aims to determine the complete DNA sequence of an organism's genome at once. This technique is significant in operon sequencing as it provides a broad view of all genetic elements, including operons. WGS is particularly beneficial because it captures not only the operons but also intergenic regions that may contain regulatory elements. The key characteristic of WGS is its ability to generate comprehensive data sets that reflect genetic diversity within a population. A unique feature of WGS is its high throughput, which allows for the analysis of multiple samples simultaneously. However, one challenge with WGS is the need for substantial computational resources to manage and interpret the large volumes of data produced.
Targeted Sequencing
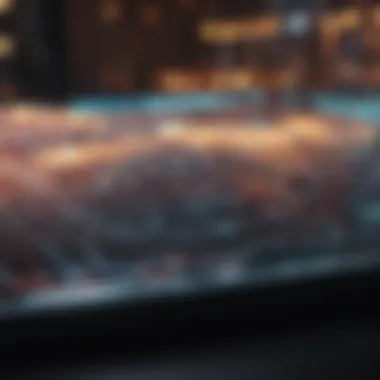
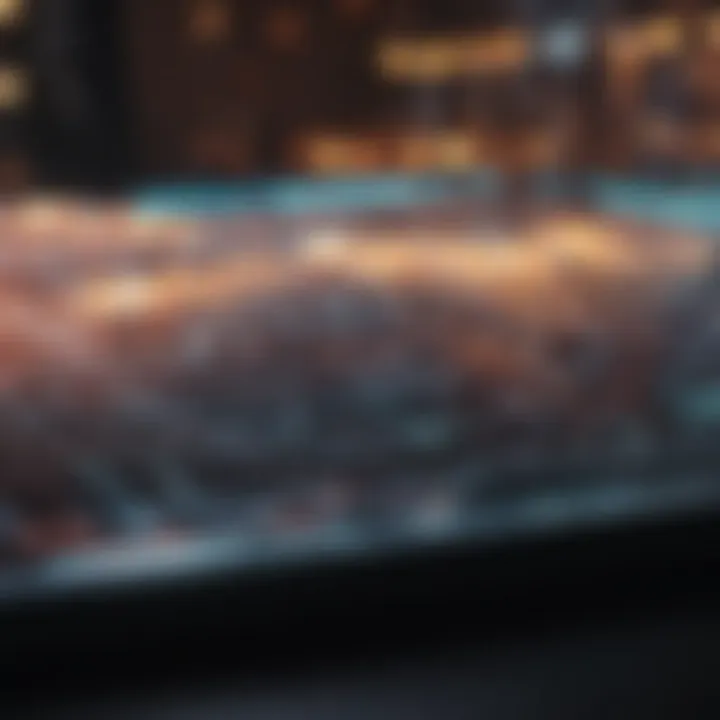
Targeted Sequencing focuses on specific regions or genes of interest, rather than sequencing an entire genome. This approach is particularly relevant for operon studies where researchers wish to drill down into specific operonic structures and their regulatory sequences. The key advantage of targeted sequencing is that it reduces the amount of data generated, allowing for more focused analysis of specific operons. This method is popular due to its cost-effectiveness and the shorter timeframe for obtaining results. One unique feature of targeted sequencing is its precision, as it enriches the specific areas of interest, thus enhancing the quality of the data obtained. However, a potential disadvantage is that it may miss critical regulatory elements found in the non-targeted regions, leading to gaps in understanding the full operonic landscape.
Data Analysis and Interpretation
Data analysis and interpretation play a critical role in operon sequencing. These processes allow researchers to derive meaningful insights from large sets of sequencing data. With advances in technology, microbiologists and geneticists can generate extensive datasets, but without robust analysis, this information remains underutilized. The ability to interpret this data leads to a clearer understanding of gene expression patterns, regulatory mechanisms, and potential functional outcomes of operons.
Bioinformatics Tools
Bioinformatics tools are essential for analyzing operon data. They provide algorithms and software capabilities to process and visualize complex datasets produced by sequencing methods. Tools such as BLAST, SAMtools, and Galaxy are frequently utilized to compare sequences and identify genes within operons. Using these tools, one can determine the structural organization of operons and analyze mutations or variations within gene sequences, which can be vital for understanding genetic regulation.
Gene Expression Analysis
Gene expression analysis is integral to assessing the functionality of operons. By measuring the levels of RNA produced, researchers can infer which genes are active under certain conditions. This analysis can occur through various methods; two prominent approaches are Quantitative Real-Time PCR and Microarray Analysis.
Quantitative Real-Time PCR
Quantitative Real-Time PCR (qPCR) is a widely respected method for gene expression analysis. Its main contribution lies in its sensitivity and specificity. qPCR quantifies RNA levels in real-time, allowing for the detection of small changes in gene expression.
The key characteristic of qPCR is its ability to provide quantifiable results in a short timeframe. It is a beneficial choice for studies focused on operons, as it can assess multiple genes simultaneously, given they are within the same operon.
A unique feature of qPCR is its incorporation of fluorescent dyes to measure the amplification process. This feature allows for a precise quantification of initial RNA amounts. However, its reliability can be affected by the quality of RNA samples and primer design, which can introduce variability in results.
Microarray Analysis
Microarray analysis is another powerful technique for gene expression analysis. This method allows for the simultaneous measurement of thousands of genes. The primary strength of microarray is its capacity to profile gene expression on a genomic scale, making it particularly advantageous for studying operons across various conditions and time points.
One key characteristic of microarray analysis is its high-throughput capacity. It can generate a vast amount of data in a single experiment, which is essential for identifying patterns in operon activity. Furthermore, microarrays can reveal interactions between different operons, providing insights into regulatory networks.
Despite its advantages, microarray analysis has limitations. These include the need for a predetermined assay design and a degree of background noise that can complicate data interpretation. Therefore, while useful, its findings must be corroborated with additional validation methods, such as qPCR.
Applications of Operon Sequencing
The study of operon sequencing transcends the realm of academic inquiry, finding practical applications across various fields, particularly in microbial genetics and synthetic biology. Understanding how genes are organized and regulated within operons is critical in deciphering genetic expressions and interactions. This is increasingly relevant as we seek to harness genetic information for practical applications.
In microbial genetics, operon sequencing plays a vital role in elucidating the complexities of gene regulation in bacteria and archaea. Traditionally, these organisms have been used as models to understand basic biological processes. With operon sequencing, researchers can identify the connections between genetic regions that co-regulate responses to environmental changes, antibiotic resistance, and virulence factors. This can lead to targeted strategies in developing antimicrobial therapies and understanding pathogenic mechanisms in various microbes.
In the context of synthetic biology, operon sequencing is essential. The ability to manipulate genetic sequences allows practitioners to design and construct novel biological parts, devices, and systems. The strategic design of operons can optimize expressions, enabling the engineering of organisms that produce valuable compounds, such as pharmaceuticals or biofuels. Each of these applications underscores the importance of operon sequencing in pushing the boundaries of genetic engineering and biotechnology.
"The manipulation of operons can lead to innovative pathways in synthetic biology, where traditional boundaries of genetic engineering are redefined."
Recognizing these applications highlights the immense potential of operon sequencing in transforming both research and industry landscapes. As technology advances, so too will the fidelity and efficiency of operon sequencing methodologies, further enhancing its role in these critical fields.
Microbial Genetics
Microbial genetics heavily relies on operon sequencing to uncover the mechanisms governing gene expression in microorganisms. By sequencing operons, scientists can dissect the regulatory elements that dictate when and how genes activate. This is paramount for understanding how these organisms adapt to their environment. For instance, when bacteria encounter stress, operons can induce the expression of genes that confer resistance.
In pathogenic microbes, operon sequencing helps identify genes that are crucial for virulence. This information can guide the development of targeted antimicrobial agents. Knowing which genes operate together provides insights into potential intervention points for controlling infectious diseases.
Synthetic Biology
Synthetic biology utilizes operon sequencing to create engineered systems for specific purposes. This is accomplished in several key areas, notably gene cloning and biomanufacturing.
Gene Cloning
Gene cloning enables researchers to replicate and manipulate specific sequences of DNA. This process is a cornerstone in synthetic biology. When it comes to cloning specific operons, it allows for the testing of hypotheses regarding genetic functions. Cloning operons can facilitate the study of gene interactions and regulatory mechanisms directly within a model organism. This approach has proven successful in enhancing the productivity of metabolic pathways, making gene cloning a beneficial choice.
One key characteristic of gene cloning is its versatility. It can be adapted to various organisms and systems. Despite challenges in ensuring the fidelity of the cloned gene's function when transferred to a different context, advancements in techniques continue improving this aspect. The benefits of efficiency and the ability to test multiple scenarios render gene cloning a principal tool in this area.
Biomanufacturing
Biomanufacturing refers to the use of living cells to produce valuable products, such as enzymes, vaccines, and biofuels. Operon sequencing plays a significant role in optimizing microbial strains for production efficiency. By understanding the operons involved in essential metabolic pathways, researchers can enhance the yield of desired products.
The key characteristic of biomanufacturing lies in its sustainability potential. It offers an alternative to traditional chemical processes, often at a lower environmental cost. The adaptability of living organisms to process various feedstocks or to tolerate adverse conditions makes biomanufacturing an attractive field. However, it also faces challenges, including scaling production processes and maintaining precision in metabolic control.
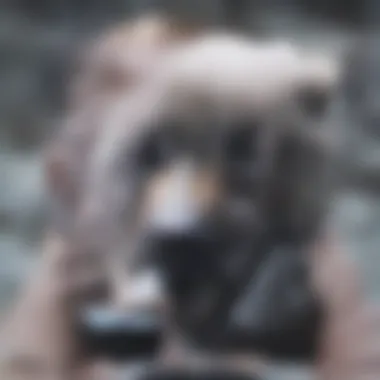
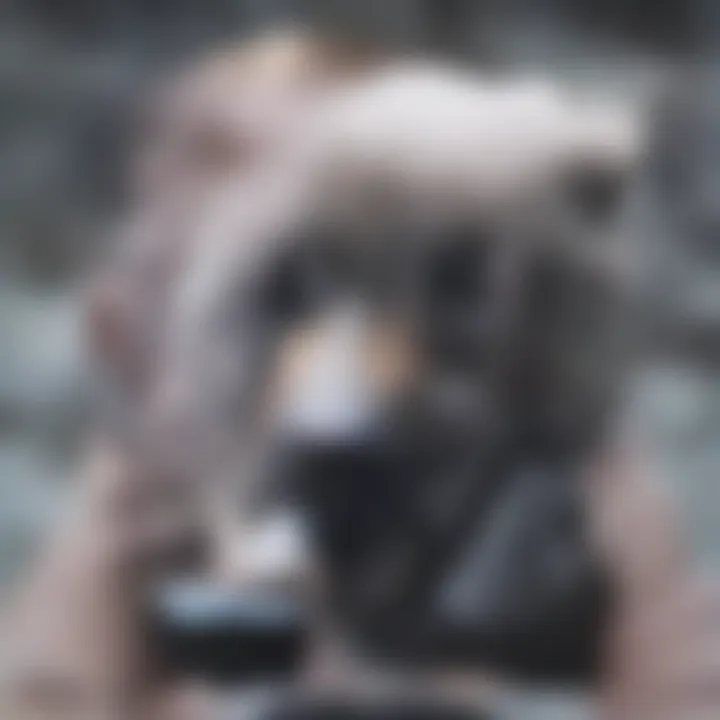
In summary, the applications of operon sequencing in microbial genetics and synthetic biology represent a significant frontier. These applications offer promising insights into genetic mechanisms and practical strategies for innovations in science and industry.
Challenges in Operon Sequencing
Operon sequencing poses a number of challenges that can hinder progress in understanding their regulatory mechanisms. Addressing these obstacles is crucial for advancing the field. The consequences of failing to thoroughly explore these challenges are significant, impacting both the comprehension of gene regulation and its practical applications in biotechnology and medicine.
Technical Limitations
One of the primary technical limitations in operon sequencing includes the complexities associated with DNA extraction. Isolating high-quality DNA from microbial cells can be problematic. Unlike the eukaryotic cells, which typically have robust cell membranes, prokaryotic cells pose unique challenges. Their diverse cell wall structures often require special techniques to lyse the cells effectively without compromising the DNA integrity.
Sequencing approaches, such as Next-Generation Sequencing (NGS), also encounter challenges. Read length and accuracy can affect the quality of the data obtained. Short reads may lead to ambiguities in sequence assembly, hindering accurate identification of operons. Additionally, high-throughput sequencing generates a significant amount of data that needs to be processed efficiently. Managing this big data often requires advanced bioinformatics tools, which may not always be accessible to all researchers.
Interpretational Difficulties
Even after successful sequencing, researchers face interpretational difficulties. Data analysis and annotation can be problematic due to the complexity of operon structures. Operons can vary greatly between species, which complicates the comparison of functional elements across different genomes. Furthermore, understanding the functional significance of each gene within an operon can require extensive biological context that is not always readily available.
There is also the challenge of gene regulatory predictions. Many operons have regulatory elements that are not well understood, making it difficult to predict how a gene's expression might change in various conditions. Biological variability can result in different expression levels under identical conditions, complicating the interpretation of experimental results.
"Addressing technical limitations and interpretational difficulties is crucial for leveraging the full potential of operon sequencing in research."
Future Directions in Operon Research
Research into operon sequencing holds significant potential. Understanding the future directions can help refine techniques and enhance genetic comprehension. The advances in sequencing are opening doors to more efficient methodologies, impacting fields such as microbial genetics and biotechnology.
Innovative Sequencing Technologies
Innovative sequencing technologies are vital for progressing in operon research. Methods like long-read sequencing and single-cell sequencing enable scientists to analyze genetic information at an unprecedented level of detail. With long-read sequencing, researchers can capture entire operons in a single pass, enhancing the understanding of their organization and function.
Moreover, advancements in nanopore sequencing technology provide real-time data analysis. This capability is beneficial for immediate decision-making in experiments. Improved accuracy also reduces the likelihood of errors, making these technologies a promising area for future exploration.
By incorporating these innovative techniques, researchers can unravel complex genetic interactions within operons, leading to more precise genetic manipulations that could benefit areas like synthetic biology and genetic engineering.
Expanding Applications
Environmental Genomics
Environmental genomics is an emerging field that explores genetic material obtained directly from environmental samples. This approach contributes ****notably to operon research. By analyzing microbial communities in various ecosystems, scientists gain insights into genetic regulations influencing biogeochemical cycles.
The key characteristic of environmental genomics is its capacity to capture a wide array of genetic diversity without the need for culturing organisms in the laboratory. This non-culturing method allows researchers to study previously uncultured microorganisms, which often play significant roles in their environments.
The advantages of this approach include improving ecosystem management and understanding microbial dynamics. However, challenges persist. Data interpretation can become difficult due to the vast amounts of information, requiring robust bioinformatics tools to analyze results effectively.
Human Health
Human health is another area where operon sequencing research is crucial. It links genetic expressions in operons to diseases and health outcomes. For instance, understanding how operons function in pathogenic bacteria can inform antibiotic development.
The key characteristic of human health research in the context of operon sequencing is its direct applicability to healthcare. By identifying specific genes within operons related to disease mechanisms, targeted therapies can be developed.
A unique feature of this focus is the potential for personalized medicine, where treatments are tailored based on an individual's genetic profile. However, challenges arise in ensuring the ethical use of genetic information and maintaining patient privacy.
Overall, both environmental genomics and human health represent vital areas for future operon research. They offer the potential for significant advancements in our understanding of genetics and its applications in solving real-world issues.
Finale
In this article, we have explored the multifaceted domain of operon sequencing, shedding light on its mechanisms and wider implications. Understanding operons is crucial for grasping how genes are regulated and expressed in both prokaryotic and eukaryotic organisms. The conclusion integrates key elements that underscore the pivotal role operons play in genetic research and biotechnology.
Summary of Key Points
Operon sequencing serves as a cornerstone in molecular biology. Here are the fundamental insights that emerged:
- Definition: Operons are units of genetic regulation that comprise a promoter, operator, and structural genes. They allow for coordinated expression of genes in response to environmental cues.
- Regulatory Mechanisms: The regulation can be transcriptional or post-transcriptional, with factors such as RNA polymerase and various transcription factors playing vital roles.
- Sequencing Techniques: Next-Generation Sequencing and targeted sequencing are vital methodologies for studying operons. They provide insights into the sequencing of gene structures and their interactions.
- Applications: The implications of operon sequencing extend into areas like microbial genetics and synthetic biology, highlighting the relevance of operons in genetic manipulation and biopharmaceutical production.
- Challenges: Technical limitations and interpretational difficulties present barriers that need to be addressed for further advancements in operon research.
Implications for Future Research
As science progresses, the understanding of operons will continue to evolve. Future research directions may include:
- Innovative Sequencing Technologies: The development of more efficient and cost-effective sequencing technologies can enhance our ability to decode complex operons.
- Environmental Genomics: Operon sequencing can uncover how organisms adapt to different environments, which is essential for ecological studies and bioremediation strategies.
- Human Health: Analysis of operons can reveal important insights into microbial communities in the human body, which may lead to advancements in personalized medicine and microbiome research.
In summary, operon sequencing stands as a powerful tool in unraveling genetic mysteries, with significant potential to transform various fields. Continuous exploration and refinement will likely yield transformative insights that can improve our understanding of genetics.